Successful Generation of 1.7-Cycle Intense Pulses at High Repetition Rate of 1 MHz~towards the next-generation bright attosecond pulse sources~
T. Okamoto1, Y. Kunihashi1, Y. Shinohara1, H. Sanada1, M-C. Chen2, K. Oguri1
1 NTT Basic Research Laboratories, Japan
2 Institute of Photonics Technologies, National Tsing Hua University, Taiwan
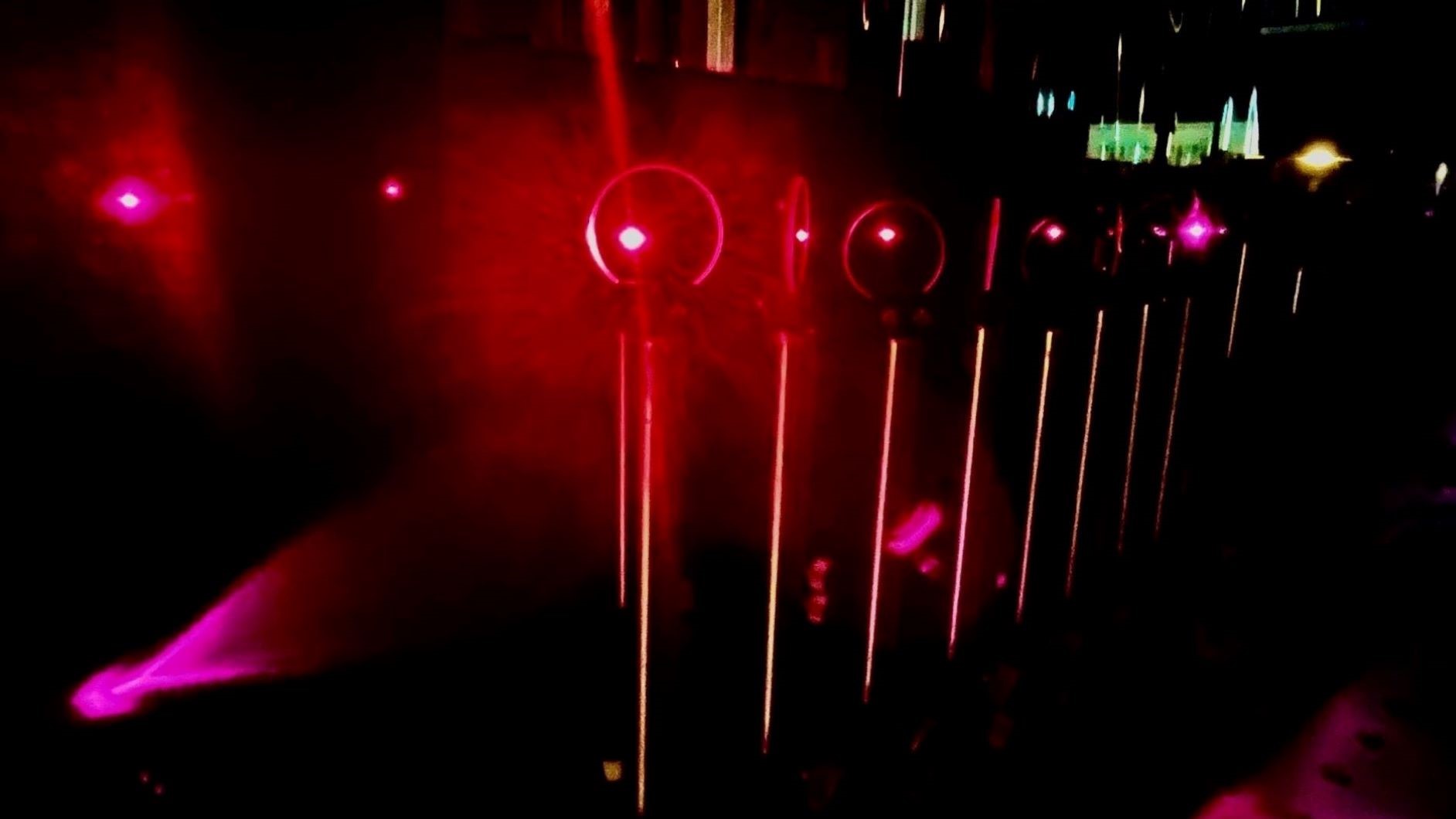
Intense pulse sources with a pulse duration of only 1.7 optical cycles has been successfully generated at very high repetition rate*1 (1 MHz = 100,000 times per second, several hundred times faster than conventional rates). It has been realized by applying a technique called "multiple-plate compression*2" to ytterbium (Yb)-based laser pulses, and possesses top-class performance despite its simple setup without large-scale laser systems and somplicated cooling systems. Since it is expected to enable the more convenient and low-cost use of high-repetition, few-cycleintense pulse sources at the MHz level previously only generated in limited large facilities is available for large number of users. Therefore, our work brings impact to various research fields including ultrafast phenomena and high-field physics.
Intense laser pulses have been usable after the emergence of the chirped pulse amplification *3, which was awarded the 2018 Nobel Prize in Physics. Today, such intense pulses are sometimes compressed for further increaing the power, and applied to numerous applications in strong-field physics, with particular emphasis placed on the generation of ultimately short pulses through high harmonic generation*4. These ultimately short pulses are known as attosecond pulses, which flickers for an extremely brief time of attoseconds (one quintillionth of a second, 10-18 seconds) and corresponds to the "flash of a camera" with the shortest exposure time ever possessed by humankind. The Quantum Optical Device Research Group at NTT Basic Research Laboratories has been dedicated to the observation of unexplored ultrafast phenomena using attosecond pulses and possesses world-class techniques in this field. In 2014, we successfully observed dynamic of inner-shell electrons. In 2016 and 2018, they found the highest-frequency response in solid materials.
As described above, few-cycle intense pulses generate attosecond pulses through high-order harmonic generation. However, the conversion efficiency of attosecond pulse generation is very low, ranging from 0.01 to 0.0001%, resulting in a very weak output of attosecond pulses. This low intensity of the attosecond pulses sometimes necessitates measurements lasting more than a day or even a week to obtain a sufficient signal. Conducting measurements under various conditions becomes impractical due to the substantial amount of time required. If this issue can be addressed, it would not only unveil previously hidden ultrafast phenomena obscured by noise, but also pave the way for new optical technologies such as groundbreaking video-rate imaging with the highest frame rate of attosecond.
In this study, we successfully generated intense pulses with a duration of only 1.7 cycles (optical periods) at a MHz repetition rate by compressing pulses from ytterbium (Yb) amplifiers*6. Conventionally, intense pulses are generated using titanium-sapphire amplifiers*7 operating at kHz repetition rates (1,000 pulses per second, 103 Hz). However, in this study, we utilized Yb amplifiers capable of MHz operation. While it offers the advantage of achieving high average power (~100 W), there is a drawback of longer pulse duration compared to titanium-sapphire amplifiers. By compressing the pulse duration, the power within a shorter time can be comcentrated, which opens possibilities for strong-field applications such as attosecond pulse generation. Therefore, we compressed the Yb laser pulses by a solid-based compression technique called "multiple-plate compression" composed of thin glass plates. By effectively using the strong response of the solid materials, we developed a very compact and simple setup compared to the conventional gas-based hollow fiber compressor*8 (see Fig. 2). With this system, we successfully generated intense pulses with a duration of only 1.7 cycles (optical periods), as depicted in Fig. 3. These 1.7-cycle intense pulses can reach an intensity of 710 TW/cm2, exceeding the required intensity (100 TW/cm2) for attosecond pulse generation, thereby demonstrating the applicability of attosecond pulse generation. Our achievement paves the way for the realization of high-repetition-rate attosecond pulse light sources.
The next objective is to develop attosecond pulse generation at MHz repetition rates using this light source, aiming to pioneer groundbreaking attosecond imaging techniques. Additionally, we aim to complement large-scale facilities such as synchrotrons and free-electron lasers by harnessing such bright attosecond pulse sources.
Terminology
- repetition rate The number of pulse occurrences per second at a fixed interval. For example, if 10 pulses occur in one second, the repetition rate is 10 Hz.
- multiple-plate compression A pulse-compression method achieved by optimally arranging thin transparent solid plates (in this study, glass was used). While the conventional compression method utilizes gases, solid-based compression offers the advantage of a stronger response due to the higher density of atoms in solids, enabling a compact setup.
- chirped pulse amplification A scheme for amplifying pulses. The weak seed pulses are temporarily stretched in time, then their intensity is amplified. Finally, the stretched and amplified pulses are compressed, resulting in intense pulses.
- high-harmonic generation When intense pulses are focused on a medium, such as a noble gas, they generate light with shorter wavelengths that have frequency components that are integer multiples of the fundamental pulses. This phenomenon, which can reach high orders such as several tens of times the frequency of the fundamental pulses, is called "high-harmonic generation."
- attosecond pulses Pulsed light with a duration in the attosecond range (100 quintillionths of a second, 10^-18 seconds), which is generated through high-harmonic generation. These pulses typically have wavelengths ranging from extreme ultraviolet to soft X-ray. By utilizing attosecond lasers as "flash of a camera", we can observe ultrafast phenomena on the attosecond timescale. Attosecond pulses represent the fastest technologies available to us and are extensively studied worldwide.
- ytterbium (Yb)-based amplifier A laser amplifier that uses laser crystals doped with ytterbium (Yb). In this study, an Yb:KGW amplifier was used, which added Yb to potassium gadolinium tungstate crystals (KGW). Its characteristic is efficient generation with reduced heat generation, allowing it to operate at high outputs exceeding 100 W.
- titanium-sapphire amplifier A laser amplifier that has been widely used for a long time to obtain intense pulses. It employs a laser medium consisting of sapphire crystals doped with titanium. The advantage is very short pulse duration, while the limitation of operation at only up to kHz repetition rates.
- hollow-core fiber compression A gas-based pulse compression method that has been extensively utilized. Since gases have relatively weak responses to light, pulses must be introduced into a long hollow fiber enclosing gas to achieve long-distance interaction.
- TW/cm2 (Terawatt per square centimeter) A unit that indicates how much power is concentrated on an area of 1 cm × 1 cm. TW represents terawatts (10^12 or 100 billion) as an SI prefix for power (W, watts). Therefore, 1 TW/cm2 indicates that 100 billion watts of light is concentrated on an area of 1 cm × 1 cm. When irradiating matter with power exceeding 100 TW/cm2, electrons orbiting around atomic nuclei are stripped off. Attosecond light is generated by these stripped-off electrons, making a laser with high intensity exceeding 100 TW/cm2 essential for attosecond pulse generation.